Titanium foam
Titanium foams exhibit high specific strength, high energy absorption, excellent corrosion resistance and biocompatibility. These materials are ideally suited for applications within the aerospace industry.[1][2][3] An inherent resistance to corrosion allows the foam to be a desirable candidate for various filtering applications.[4][5] Further, titanium’s physiological inertness makes its porous form a promising candidate for biomedical implantation devices.[6][7][8][9][10][11] The largest advantage in fabricating titanium foams is that the mechanical and functional properties can be adjusted through manufacturing manipulations that vary porosity and cell morphology. The high appeal of titanium foams is directly correlated to a multi-industry demand for advancement in this technology.
Characterization
Banhart [12] describes two dominating perspectives in which cellular metals are characterized, referring to them as atomistic and macroscopic. The atomistic (or molecular) perspective holds that a cellular material is a construction of struts, membranes, and other elements which possess mechanical properties of their bulk metal counterpart. Indeed, the physical, mechanical, and thermal properties of titanium foams are commonly measured using the same methods as that of their solid counterparts. However, special precautions must be taken due to the cellular structure of metal foams.[13] From a macroscopic perspective, the cellular structure is perceived as a homogenous structure and characterized by considering the effective (or averaged) material parameters.[12]
Mechanical properties
As with other metal foams, the properties of titanium foams depend mostly on the properties of the starting material and the relative density of the resultant foam.[14] Thermal properties in foams – such as melting point, specific heat and expansion coefficient – remain constant for both the foams and the metals from which they are composed. However, the mechanical properties of foams are greatly influenced by microstructure, which include the aforementioned properties as well as anisotropy and defects within the foam’s structure.[15]
Sensitivity to impurities
The mechanical properties of titanium foams are sensitive to the presence of interstitial solutes, which present limitations to processing routes and utilization. Titanium has a high affinity for atmospheric gases. In foams, this is evidenced by the metal’s tendency to trap oxides within cell edges.[16][17][18] Micro-hardness of cell walls, elastic modulus, and yield strength increase as a result of interstitial solutes; ductility, which is a function of the quantity of interstitial impurities, is consequently reduced.[19][20] Of the atmospheric gases, nitrogen has the most significant impact, followed by oxygen and carbon.[21] These impurities are often present in the precursor mixture and also introduced during processing.
Theoretical models for predicting mechanical properties
Gibson & Ashby models
Gibson & Ashby [22] micromechanical models for porous materials provide mathematical equations for the prediction of mechanical parameters based on experimentally determined geometric constants. The constants of proportionality are determined by fitting experimental data to various mathematical models for structures consisting of cubes and solid struts and are dependent upon cell geometry. A limitation of the Gibson & Ashby [22] model is that it is most accurate for foams exhibiting porosities higher than 70%, although experimental comparisons for lower porosity foams have shown agreement with this model. Ye & Dunand[23] found reasonable agreement to the Gibson & Ashby model for titanium foams exhibiting 42% porosity. Ultrasonic measurements provided an average Young’s modulus value of 39 GPa, which is in relatively good agreement with the Gibson & Ashby prediction of 35 GPa.
The Gibson & Ashby[22] models assume ideal structures; microstructural irregularities (e.g. inhomogeneous pore distribution; defects) are not considered. Additionally, experimental results from which the predetermined proportionality constants were based on experimental values that were obtained from simple compression tests. Consequently, they may not be applicable for multiaxial loads.[24] Overall, the models are an excellent tool for general estimations of mechanical parameters; yet, precise predictive values cannot be relied upon
Minimum solid area (MSA) models
Minimum solid area models assume that the load bearing area (cross-sectional area normal to the stress) is the logical basis for modeling mechanical behavior.[25] MSA models assume pore interaction results in reduction of stress. Therefore, the minimum solid areas are the carriers of stress. As a result, predicted mechanical properties fluctuate based on the quantification of the solid area of the foam.[26] For titanium foams consisting of partially sintered powders, the minimum solid area consists of the neck area between powders through the cross-section of cell walls between macropores.[27] The mathematical relationships in MSA models[28] are relatively consistent with the Gibson & Ashby[22] model.[29] However, the MSA models are designed to predict mechanical property parameters over a broader range of porosity levels. Like the Gibson & Ashby models, MSA models were derived assuming idealized (defect-free) structures containing uniform pore shapes, size and distribution.
Compressive properties
The most frequently reported mechanical property of titanium foams is compressive strength.[30] It was generally accepted that the compressive properties of metal foams depended on the properties of the cell wall rather than on pore size. However, more recent research has indicated that smaller pore sizes equate to higher compressive strength. As pore sizes reach nano-dimensions, the relationship is even more clear due to changes in deformation mechanism.[31] Tuncer & Arslan[32] fabricated titanium foams via the space-holder method using various shaped space-holders to elucidate the effect of cell morphology on mechanical properties. They found that foams created with needle-like, carbamide space-holders exhibited a decrease in elastic modulus and yield strength when compared to spherical pores.[32]
Here all the credit goes to the Guruji of my Eklavya.
Processing methods
Many metal foam manufacturing techniques are accomplished by the introduction of a gaseous phase into a precursor matrix, which can occur in either molten metal or a powdered metal form. Due to titanium's high melting point (1670 °C) and high chemical affinity with oxygen, nitrogen, carbon and hydrogen (which dissolve rapidly either in liquid or solid titanium at a temperature above 400 °C[17]), solid-state processes based on powder densification are the preferred method of fabrication.[23][17][27][30][33][34] Processing methods must also be designed to avoid exposure to air or moisture; vacuum or inert gas sintering processes are usually sufficient for preventing contamination.[17][35]
Loose-powder (gravity) sintering


Utilizing powder metallurgy routes[36] for titanium foam fabrication allows for production at lower temperatures than those required through a melt process and reduces overall risks for contamination. In loose-powder sintering (also known as gravity sintering), pores are created through diffusion bonding arising from the voids existing between packed powder particles. Axial compaction followed by sintering follows the same procedure as above, but pressures is applied for compaction of the precursor material.[37] For both compaction methods, the resulting pore morphology is dependent upon the morphology of the metallic powder, making it difficult to control the size, shape, and distribution of the pores.[36] Another disadvantage includes the relatively high probability of pore collapse and limited achievable porosity levels.[38][39]
Expansion of pressurized bubbles

To produce titanium foams via expansion of pressurized gas, the titanium precursor mixture is placed within a gas-tight metal can, which is evacuated after filling. The metal can is pressurized with inert gas—most commonly argon – and is pressed isostatically. The gas-filled pores are contained within the compacted matrix, and upon exposure to elevated temperatures, these bubbles expand through creep of the surrounding metal matrix.[40] Since processing titanium foams using hot isostatic pressing (HIP) eliminates the need for separate compaction and sintering processes, a wider variety of custom shapes and sizes are possible than via loose powder sintering techniques.[41] Disadvantages of this process include reduced pore connectivity, limited achievable porosity, and a complicated experimental set-up.[41] However, a unique aspect of the HIP process with respect to titanium (and other polymorphic materials) is that transformation superplasticity can be enhanced through the HIP process by way of thermal cycling, or by cycling around the alpha/beta allotropic temperature boundaries of the metal.[33]
Superplastic expansion
Titanium undergoes allotropic transformation from its α-phase (hexagonal close-packed (HCP) structure at temperatures less than 882.5 °C) to its β-phase (body centered cubic (BCC) structure at temperatures above 882.3 °C). Alpha-phase titanium products typically exhibit medium to high strength with excellent creep strength, whereas beta-phase titanium products typically exhibit very high strength and low ductility.[33][37] Foams created under thermal cycling conditions have been shown to exhibit increased porosity due to the density difference between allotropic phases. Davis, Teisen, Schuh & Dunand[33] produced titanium foams with 41% porosity (as compared to 27% porosity through the normal HIP creep mechanism). Increases in overall ductility were also observed in foams created through thermal cycling. In a similar experiment, porosity of 44% was achieved and determined as the maximum achievable porosity under thermal cycling conditions.[42] A later study also utilized exploitation of transformation superplasticity conditions through HIP, but in this case, the titanium powder in the precursor matrix was replaced with titanium wires to create anisotropic pores. The resulting anisotropic pores showed closer correlation with natural bone in that the foams exhibited higher elastic moduli, yield strength and deformation when subjected to longitudinally loaded forces than when loads were applied transversely.[43]
Space-holder technique

The space-holder technique is the most commonly employed method for producing titanium foams.[19][27][31][32][41][44][45][46] [47] [48] [49][50][51][52][53][54] [55] [56] [57] [58] [59] [60][61][62] [63] [64] [65] [66][67] [68] [69] [70] [71] [72] [73] [74] [75] [76] [77] [78] [79] [80] [81] [82] The space-holder technique allows for the fabrication of higher porosity foams (35-80% [83]) than other techniques, while also giving the engineer more control over pore fraction, shape and connectivity.[40] Mechanical properties can be adjusted through the size, shape and quantity of space-holders employed. The space-holder technique was first demonstrated by Zhao and Sun[84] for the fabrication of aluminum foams in a powder metallurgical method, which consisted of the incorporation of NaCl as a space-holder. The space-holder was mixed into the powder mixture and dissolved prior to sintering. The same method was used to create titanium foams for the first time when Wen et al [80] utilized ammonium hydrogen carbonate spacers.
Powder selection
The size and shape of the metal powder has a direct impact on the stability of the precursor as well as the resulting foam. For this purpose, powders that increase packing efficiency are most advantageous.[32] The use of spherical particles may result in less contact of particles which consequently leads to larger secondary pores and a higher probability of pore collapse prior to complete sintering.[85] This factor can be limited through different compaction techniques that decrease the degree of interstitial sites around the titanium particles. However, this method also has limitations; for example, the powders cannot be compacted to such a degree that would promote deformation of the spacer (unless anisotropic pore shape is desired).[23][54][86]
Space-holder selection

The selection of the space-holder is one of the most crucial steps because it defines many of the properties of the resulting foam, including cell shape, cell size and macroporosity. The space-holder should be inert and represent the size and shape of the desired pores. The porosity may be adjusted anywhere between 50 and 85% without the filler material becoming a part of the resultant foam.[10] It is also important to select a spacer that has limited or no solubility in titanium, as this incorporation will affect the mechanical properties of the resulting foam.[87] It is also possible to produce gradient structures in which the porosities differ within the foam based on the differences in spacer size in the preform.[86]
Space-holder size and shape
The degree of homogeneity in pore distribution of the final product is primarily dependent on the adequacy of mixing of the precursor. The difference in particle size between the titanium powders and the spacers directly impacts the ability to adequately mix the preform. The greater the size difference, the more difficult it is to control this process.[87] Nonhomogeneous mixing resulting from the use of spacers considerably larger than the titanium particles employed and has shown adverse effects in the stability of the precursor after removal of spacer and in the distribution of porosity.[32][38][88] Spacer size has been investigated by Tuncer & Sharma in three different studies.[32][41][78] It was shown that the use of a coarse spacer results in thicker pore walls while the use of finer spacers results in enhanced compaction, leading to increased densification. Increased densification is evidenced by a monomodal pore distribution with the employment of fine spacers and a bimodal distribution using coarse spacers. Further, finer spacers result in a more homogenous pore distribution. Sharma et al [70] utilized acicular spacers and achieved porosities up to 60% where pores were undistorted. In samples employing fine particles, porosities up to 70% were achievable before noting distortion in the pores.[78] However, the bimodal pore distribution observed in coarse-spacer samples showed to be beneficial in terms of mechanical properties in that higher compressive strengths were observed, beyond those that might exist due to the inverse relationship of porosity and compressive strength alone.[78]
Compaction
The precursor mixture of powders and space-holders are compacted into a mold under a specified pressure. This can be achieved through uniaxial or isostatic processes. The pores resulting from this method are open and interconnected via windows between neighboring pores with the size of the pores partially dependent upon the coordination number and contact area of the resulting compact. Compaction pressure must be high enough to ensure sufficient mechanical strength for retention of pore geometry specified by the space-holder, but not too high enough as to cause deformation of the space-holder.[87]
Sintering and space-holder removal
Spacers can be categorized by their mode of removal: those which are removed thermally when temperature is raised during sintering and those which are dissolved with a solvent, either prior to or after foam formation.[86] For spacers removed via thermal mechanisms, the sintering temperature should be high enough to decompose the spacer, but not high enough to induce diffusional bonding between the metal particles. This results in a very fragile compact prior to higher temperature sintering and increases risk of collapse.[86] When employing dissolvable spacers, it is possible to remove the spacer after sintering, which reduces the risk of pore collapse. In most cases, foams created using space-holders contain bimodal pore distributions with macro-sized pores resulting from the space-holder particles and micro-sized pores located on the pore walls and resulting from incomplete sintering of the powder matrix. As a result, the macropores typically exhibit rough internal surfaces.[89] In some applications, such as for the use of bio-medical implants, this is an advantageous property. Inner porosity (or micro-porosity) has been shown to reduce stiffness; thus, reduce the risk of stress-shielding effects, while also offering improved osseointegration.[90][70][89]
Space-holder materials
Sodium chloride (NaCl)
Sodium chloride is the most commonly chosen space-holder for titanium foams[19][66][68][79][81][82][91] [92] [93] [94][95] because it is highly soluble in water and inert with respect to titanium. This inertness prevents contamination and degradation of the mechanical properties of the resulting foam. Moreover, NaCl is non-toxic; any residuals are bioinert.[70][73]
Bansiddhi & Dunand [96] first demonstrated NaCl as a permanent space-holder for the fabrication of NiTi foams. The resulting foams consisted of 32-36% porosity with more complete densification than they observed when producing NiTi foams using a sodium fluoride (NaF) space-holder.[97] However, processing parameters resulted in molten NaCl and a metal/salt blend in the cavities of the foam. Certain risks are associated with using a molten space-holder including reaction with the metal, dissolving of the space-holder in the metal and prevention of densification through the creation of a thin layer of liquid between the metal and particles.[89] Near complete densification was achieved when NaCl was used as a permanent space-holder in pure titanium foam.[23] In this case, a temperature below the melting point of NaCl was used; titanium is less creep resistant than NiTi, which allows for densification at lower temperatures. The resulting foams achieved porosity of 50-67% with minimal observable microporosity. Anisotropic pore shape in some areas alluded to NaCl’s deformation during HIP, which is desirable for some applications.[81] Additionally, an observed, rough inner surface of the pores holds advantages for biomedical implant applications. Jha et al.[85] achieved 65-80% porosity through the use of NaCl as a space-holder and a cold compaction process at various pressures with two-stage sintering. In this case, NaCl was removed through dissolution after the second stage of sintering. Resulting Young’s moduli (8-15 GPa) were considerably lower than the Young’s modulus of 29 GPa achieved by Ye & Dunand for 50% porosity foams.[19][81] This illustrates the known relationship between porosity and Young’s modulus wherein Young’s modulus decreases linearly with increasing porosity. Achievable porosity through the space-holder method is directly related to the type and amount of space-holder utilized (up to a threshold maximum achievable porosity level).
Magnesium
Magnesium can be removed either thermally or by reactive measures through the dissolution in acid.[27][98] [99] Esen & Bor [27] found a critical content of magnesium as a space holder to be 55-60%, above which compacts shrink excessively during sintering. Foams ranging in porosity from 45 to 70% with a bimodal pore distribution and compressive strength of 15 MPa (for 70% porosity) were demonstrated. Kim et al.,[99] fabricated foams with anisotropic pores through the intentional deformation of Mg particles during compaction in an effort to enhance mechanical properties. A final porosity of 70% equated to a yield strength of 38 MPa for normal orientation of pores and 59 MPa when pores were aligned with the direction of compression.
Carbamide (urea)
Another commonly employed space-holder for titanium foams is carbamide; demonstrated porosities range from 20-75%.[32][68][70][77][78][80][100] Wen et al [80] produced foams exhibiting a bimodal pore distribution with porosities ranging from 55-75%, Young’s moduli between 3-6.4 GPa, and a plateau stress of 10-35 MPa. An inverse relationship between plateau stress and porosity was observed with increased porosity resulting in decreased plateau stress.[80] Tuncer et al [77] utilized carbamide in combination with irregular shaped titanium powders in an effort to increase green strength through increased packing efficiency (of particles). This also eliminated the need for the incorporation of a binder.
Tapioca starch
Tapioca starch can be burnt off easily through the sintering process and is insoluble in titanium. Titanium foams consisting of a bimodal pore distribution (macropores ranging from 100-300 μm) and 64-79% porosity, exhibited yield strengths of 23-41 MPa and Young's moduli of 1.6-3.7 GPa.[65]
Ammonium bicarbonate
Although ammonium bicarbonate has been utilized in the manufacturing of titanium foams,[56][72][80] it is not an ideal spacer in that it has a low melting/dissociation point and some solubility in titanium.[60] This results in considerable shrinkage which makes control of pore shape difficult. Furthermore, the decomposition releases environmentally harmful gases.[44]
Freeze-casting
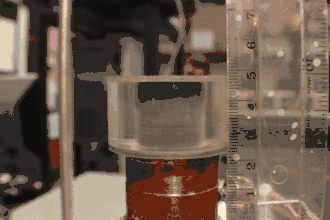

Freeze-casting is a directional solidification technique that is utilized to fabricate materials exhibiting anisotropic, elongated pore structures.[101][102] Pore morphology is defined, in large part, by the morphology of the solidified fluid. Titanium foams exhibiting dendritic[103][104] [105] [106][107][108] and lamellar[109][110][111][112] pore structures have been produced, through the use of non-aqueous and aqueous processing respectively. These materials exhibit anisotropic mechanical properties as a result of their anisotropic pore structures. Compressive strength for loads applied parallel to the wall direction of titanium foams are found to be, on average, 2.5 times greater than for those applied perpendicular to the wall direction.[102]
Applications
Potential structural applications for titanium foams include their general incorporation into light-weight structures and as components for mechanical energy absorption. The most important considerations for the use of titanium foams in structural applications includes their porosity, specific strength, ductility in compression and cost. Because of low manufacturing costs, most metal foams marketed for structural applications are of a close-celled aluminum variety.[113] In comparison, titanium foam manufacturing incurs a higher cost, but this cost is defensible in space applications where the material offers an otherwise incomparable reduction in overall weight. The lower thermal conductivity of titanium may also be appreciated in rocket construction.[1] The specific strength, overall energy absorbing capability and high melting point all reinforce titanium’s superiority to aluminum in aerospace and military applications.[3] When used for aerospace applications, levels of porosity close to 90% are desired.[73] Titanium foams are capable of retaining their high tensile strength at temperatures up to 400 °C; a limit imposed by the metal’s low resistance to oxidation.[37]
Aerospace applications
The driving force for titanium foam’s replacement of existing materials in the aerospace sector results from the following five factors:[37]
• Weight reduction: as a substitute for steels and nickel-based superalloys;
• Application temperature: as a substitute for aluminum and nickel-based alloys and steels
• Corrosion resistance: as a substitute for aluminum alloys and low-alloyed steels
• Galvanic compatibility: with polymer matrix composites as substitutes for aluminum alloys
• Space constraints: as substitutes for aluminum alloys and steels
The most urgent problem of engineering and its advanced branch of aerospace engineering is the efficient use of materials as well as increased service life.[1]
Sandwich panel cores
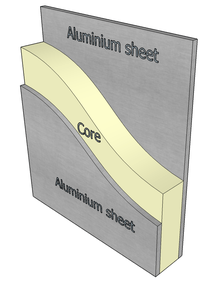
Sandwich panel cores are used throughout the aerospace industry; they are integrated within aircraft bodies, floors and internal panels. Sandwich constructions consist of two faces separated by a thick, light-weight core and are most commonly composed of balsa-wood, foamed polymers, glue-bonded aluminum or Nomex (paper) honeycombs. Typically, the cores are combined with reinforcing fibers to increase their shear modulus.[114] Indeed, carbon fiber-reinforced polymers exhibit the highest specific stiffness and strength of these materials.[115][116] However, polymers decompose at low temperatures; thus employment of the aforementioned materials pose inherent challenges due to the limited range of temperature they may be utilized within as well as their moisture-dependent properties.[13] The largest and most inadequately predicted failure within the core results from strain localization. Strain localization refers to the development of bands exhibiting intensive straining as a result of the localization of deformations in the solid.[117] [118] For the best performance, the structure should exhibit low peak response force and high total energy absorption.[119] Titanium foams are lightweight, stiff, and possess the capability to resist blast. Furthermore, the use of titanium-based foams exhibiting homogenous porosity distribution would significantly decrease the risks associated with strain localization. The high strength-to-weight ratio of titanium foams offers an opportunity to provide increased bending and shearing stiffness as well as energy absorption capabilities during periods of bending.[114][118][120] Titanium foams may be utilized in environments with elevated temperatures (up to 400 °C). Composite structures may also be produced; the incorporation of silicon carbide monofilaments into Ti-6-Al-4V foams was shown to exhibit an elastic modulus of 195 GPa and tensile strength of 800 MPa.[121]
Auxetic structures
Titanium foams exhibiting auxetic pore structures are of interest for incorporation in sandwich panel cores due to their enhanced shear performance.[122] [123] [124] Foams with this pore structure exhibit negative Poisson’s ratio in one or more dimensions.[114] Poisson’s ratio is defined as the ratio of the lateral contractile strain to the longitudinal tensile strain for the foam undergoing uniaxial tension in the loading direction.[125] Auxetic materials are typically able to resist indentations through their response to compression; upon compression, the auxetic material contracts.[125] In addition to indentation resistance, research has shown that auxetic foams offer better absorption of sound and vibration, enhanced shear resistance and facture toughness. These structures also exhibit synclastic bending, which results lends itself to integration within curved sandwich panels.
Biomedical implants


Titanium alloys are the choice material for a diverse range of biomedical implants.[126] Currently employed titanium alloy implants include: hip joints,[127] bone screws,[9][128] knee joints,[89][129] spinal fusions,[8] shoulder joints,[89] and bone plates.[126][130][131] These alloys range from high ductility, commercially-pure titanium foams with high formability, to heat-treatable alloys with high strength.[132][133] Titanium is well-suited for use in Magnetic Resonance Imaging (MRI) and Computed Tomography (CT),[134] [135] [136] which further enhances its applicability for biomedical implant applications.
Bioimplants: Microstructure
Biomedical implants should have low density for patient comfort and high porosity and surface area to facilitate vascularization and the ingrowth of new bone.[137] Ideally, the implant will allow sufficiently easy fluid flow for cell nutrition and osteoblast multiplication as well as migration for cellular colonization of the implant to become uniform. The pores contained within the foam’s cellular matrix mimic the extracellular matrix of bone, allowing the body to fixate with the implant. The porosity of the implant also promotes apposition and facilitates vascularization−as cells are able to attach, reproduce and form basic functions.[79] It has been shown that a macropore size of 200-500 µm is preferred for ingrowths of new bone tissues and transportation of body fluids. The lower bound is controlled by the size of cells (~20 µm), and the upper bound is related to the specific surface area through the availability of binding sites.[79] Finer pores further help in tissue growth and biofluid movement.[138] Anisotropic, elongated pores (such as those attainable via the freeze-casting technique) may be beneficial in bone implants in that they can further mimic the structure of bone.
The porous surface geometry of the foam promotes bone in-growth, provides anchorage for fixation, and ensures stresses are transferred from the implant to the bone.[139] Surface roughness in the pore can enhance bone in-growth, and coarser cell size facilitates faster tissue growth.[81] To optimize the implant's functionality and ability to successfully fuse with bone, it may be necessary to manipulate the material's manufacturing methods in order to modify the foam's pore structure. Changes in pore structure can directly influence implant strength as well as other key properties.
Bioimplants: Mechanical properties
Human cancellous bone possesses a stiffness ranging from 12-23 GPa;[140] careful control and modification of manufacturing parameters to achieve similar strengths is imperative for practicality of integration.[141] Correctly predicting the Young’s modulus for foams is imperative for actual biomedical integration; a mismatch of Young’s moduli between the implant and the bone can result in stress-shielding effects from a disproportional handling of stress.[142] The implant which typically exhibits a higher Young’s modulus than the bone will absorb most of the load. As a result of this imbalance, the starting bone density will be reduced, there will be tissue death and, eventually, implant failure.[143]
Natural bone exhibits the ability to adjust local fiber away from the low-stress regions toward high stress regions through the distribution of porosity, thus maximizing overall comfort.[144] Using finite element analysis, researchers examined the effect of filling pores with bone on mechanical properties.[143] They concluded that bone ingrowth significantly improved the mechanical properties, evidenced by decreased localized plasticity and stress concentrations. In effect, the titanium foam in the study allowed the bone to exhibit its natural ability to adjust local fiber away from the low-stress regions toward high stress regions.
Previous experimentation and analysis has demonstrated that random combinations of pore size and shape result in lower Young’s moduli. Theoretical models for the quantification of Young’s moduli do not account for random pore size and shape distribution, so experimental measurements must be conducted in the presence of heterogeneous pore size and distribution. This is a limitation of the micro-mechanical models discussed above.
Osseointegration
Currently utilized implants take a great deal of time to integrate with the body after the initial surgical procedure occurs. True adhesion between the implant and the bone has been difficult to achieve and, unfortunately, success rates of implant fixation are low due to the implant's failure to achieve long-term osseointegration into the bone.[88][89][145] [146] With an increasing number of individuals requiring orthopedic implants,[11] the development of materials with structural and biological potential to improve osseointegration is crucial. Utilization of titanium-based foams present one way to potentially improve the bioactivity [6][147] [148] [149] and reduce stress-shielding effects of currently employed bioimplant materials.
The problem of osseointegration is best understood by examining the process of natural bone growth. In the body, bone and tissues experience self-regeneration, and structural modifications occur normally in response to environmental stimuli.[150] Successful osseointegration occurs in three main stages that follow a natural biologically determined procedure: (1) incorporation of the implant into the bone’s formation, (2) adaption of the new bone mass to carry weight and (3) remodeling of the new bone structure. The first stage in this process is the most crucial for overall success;[151] the implant and the bone must form a rapid connection, and this bond must be strong and enduring. Due to its porous structure, a titanium metal foam implant may be able to achieve close fixation with the bone and will decrease patient recovery time considerably. Essentially, the foam becomes an extracellular matrix in the body as tissue is integrated into it.[79] Today, the implants most commonly used for bone replacement lack the ability to promote these characteristics, which are found in natural bone and, as a result, the implants have limited lifetimes.[79] This phenomenon of osseointegration works similarly to direct fracture healing. However, instead of a bone fragment-end reconnecting to bone, the fragment-end connects to an implant surface.[151] In a study on fibroblastic interactions with high porosity Ti6Al4V, the metal foam was supportive of cell attachment and proliferation, migration through the porous network, and proved capable of sustaining a large cell population.[7]
Increasing bioactivity via coatings
Titanium’s propensity to form an oxide layer on its surface prevents corrosion of surfaces that are in contact with human tissues because the surface oxides minimize diffusion of metal ions from the bulk material to the surface.[142][152] When titanium gains a coating to make it more bioactive, it can turn the already biocompatible titanium surface into an interface able to enhance osteoblast adhesion and able to promote osseointegration.[143] Today, research is heavily focused on improving the success rate of integration and uses an understanding of the natural process of bone growth and repair to create coatings that will enhance the surface finish and surface properties of the implant. These adjustments allow the artificial structure to mimic biological materials and to gain acceptance into the body with fewer negative side effects.[153] [154] A 3-year clinical and radiographic study found implants in humans coated by nanocrystalline HA to support osseointegration. The nanocrystalline HA was developed with a large rough surface of interconnecting pores between 10 and 20 nm of the silica matrix gel, resulting in a porous bone structure. Mean rates of marginal bone loss were insignificant and the periotest values were indicative of a solid osseointegration.[155] In effect, the pores are structured in such a way that they are able hold onto the proteins on the biomaterial’s surface. Ideally, this allows the body to engage in self-repair in that the synthetic HA is recognized as a like-nanomaterial in which live tissues may develop[10]
Titanium foams can be coated with HA through various methods including plasma spraying, sol-gel and electrophoretic deposition. It has been shown that HA-coated titanium exhibits increased interfacial strength in comparison to titanium foams without the coating. In an effort to enhance bone in-growth, Spoerke et al. developed a method for growing organoapatites on titanium implants. Organoapatites may assist in-bone in-growth at the implant interface. The foams were manufactured using a modified HIP process, which exploits the allotropic nature of titanium to create higher porosity foams. Previous in vitro experimentation with the organoapatite-titanium foam held promising results including the possibility that ingrown tissue within these coated pores will improve the lifetime use of the foam through reduction of stress-shielding effects.[43]
Animal studies
In the lab, synthetic nanocrystalline bone grafting material in mice has shown in-growth of vascularized fibrous tissue which resulted in improved healing. Furthermore, new blood vessels were observed at day 5 after implantation, and the implant showed a high functional vessel density.[138] In a study examining the femoral epiphyses of rabbits in two to eight weeks of healing, bone-to-implant contact was compared to bone growth inside the chambers for four different implant surfaces. The researchers found that bone substitute materials may improve the bone apposition onto titanium.[156]
See also
External links
References
- 1 2 3 A. Ermachenko, R.Y. Lutfullin, R. Mulyukov, Advanced technologies of processing titanium alloys and their applications in industry, Rev. Adv. Mater. Sci 29 (2011) 68-82.
- ↑ K. Hurysz, J. Clark, A. Nagel, C. Hardwicke, K. Lee, J. Cochran, T. Sanders, Steel and titanium hollow sphere foams, MRS Online Proceedings Library Archive 521 (1998).
- 1 2 A. Salimon, Y. Brechet, M. Ashby, A. Greer, Potential applications for steel and titanium metal foams, Journal of materials science 40(22) (2005) 5793-5799.
- ↑ L. Gauckler, M. Waeber, C. Conti, M. Jacob-Duliere, Ceramic foam for molten metal filtration, JOM Journal of the Minerals, Metals and Materials Society 37(9) (1985) 47-50.
- ↑ L.P. Lefebvre, J. Banhart, D.C. Dunand, Porous Metals and Metallic Foams: Current Status and Recent Developments, Advanced Engineering Materials 10(9) (2008) 775-787.
- 1 2 J.R. Bush, B.K. Nayak, L.S. Nair, M.C. Gupta, C.T. Laurencin, Improved bio‐implant using ultrafast laser induced self‐assembled nanotexture in titanium, Journal of Biomedical Materials Research Part B: Applied Biomaterials 97(2) (2011) 299-305.
- 1 2 N. Cheung, I. Ferreira, M. Pariona, J. Quaresma, A. Garcia, Melt characteristics and solidification growth direction with respect to gravity affecting the interfacial heat transfer coefficient of chill castings, Materials & Design 30(9) (2009) 3592-3601.
- 1 2 F.B. Christensen, Lumbar spinal fusion. Outcome in relation to surgical methods, choice of implant and postoperative rehabilitation, Acta orthopaedica Scandinavica. Supplementum 75(313) (2004) 2-43.
- 1 2 F.B. Christensen, M. Dalstra, F. Sejling, S. Overgaard, C. Bünger, Titanium-alloy enhances bone-pedicle screw fixation: mechanical and histomorphometrical results of titanium-alloy versus stainless steel, European Spine Journal 9(2) (2000) 97-103.
- 1 2 3 R. Kanaparthy, A. Kanaparthy, The changing face of dentistry: nanotechnology, International Journal of Nanomedicine 6 (2011) 2799.
- 1 2 E.K. Simpson, R.A. James, D.A. Eitzen, R.W. Byard, Role of orthopedic implants and bone morphology in the identification of human remains, Journal of Forensic Sciences 52(2) (2007) 442-448.
- 1 2 J. Banhart, Manufacture, characterization and application of cellular metals and metal foams, Progress in materials science 46(6) (2001) 559-632.
- 1 2 M.F. Ashby, Metal foams: a design guide, Butterworth-Heinemann2000.
- ↑ M.F. Ashby, T. Evans, N.A. Fleck, J. Hutchinson, H. Wadley, L. Gibson, Metal Foams: A Design Guide: A Design Guide, Elsevier2000.
- ↑ P. Kelly, D. Nowell, Three-dimensional cracks with Dugdale-type plastic zones, International journal of fracture 106(4) (2000) 291-309.
- ↑ M. Barrabés, A. Michiardi, C. Aparicio, P. Sevilla, J.A. Planell, F.J. Gil, Oxidized nickel–titanium foams for bone reconstructions: chemical and mechanical characterization, Journal of Materials Science: Materials in Medicine 18(11) (2007) 2123-2129.
- 1 2 3 4 D.C. Dunand, Processing of titanium foams, Advanced engineering materials 6(6) (2004) 369-376.
- ↑ L.-P. Lefebvre, E. Baril, Effect of oxygen concentration and distribution on the compression properties on titanium foams, Advanced Engineering Materials 10(9) (2008) 868-876.
- 1 2 3 4 N. Jha, D. Mondal, J. Dutta Majumdar, A. Badkul, A. Jha, A. Khare, Highly porous open cell Ti-foam using NaCl as temporary space holder through powder metallurgy route, Materials & Design 47 (2013) 810-819.
- ↑ N. Dukhan, Metal foams: fundamentals and applications, DEStech Publications, Inc2013.
- ↑ J. Luyten, S. Mullens, I. Thijs, Designing with pores—synthesis and applications, KONA Powder Part J 28 (2010) 131-142.
- 1 2 3 4 L.J. Gibson, Cellular solids, Mrs Bulletin 28(4) (2003) 270-271.
- 1 2 3 4 B. Ye, D.C. Dunand, Titanium foams produced by solid-state replication of NaCl powders, Materials Science and Engineering a-Structural Materials Properties Microstructure and Processing 528(2) (2010) 691-697.
- ↑ S. Maiti, L. Gibson, M. Ashby, Deformation and energy absorption diagrams for cellular solids, Acta metallurgica 32(11) (1984) 1963-1975.
- ↑ R.W. Rice, Porosity of ceramics: Properties and applications, CRC Press1998.
- ↑ M. Scheffler, P. Colombo, Cellular ceramics: Structure, manufacturing, properties and applications, John Wiley & Sons2006.
- 1 2 3 4 5 Z. Esen, Ş. Bor, Processing of titanium foams using magnesium spacer particles, Scripta Materialia 56(5) (2007) 341-344.
- ↑ R. Rice, Evaluation and extension of physical property-porosity models based on minimum solid area, Journal of materials science 31(1) (1996) 102-118.
- ↑ R. Rice, Comparison of stress concentration versus minimum solid area based mechanical property-porosity relations, Journal of materials science 28(8) (1993) 2187-2190.
- 1 2 M.M. Shbeh, R. Goodall, Open Celled Porous Titanium, Advanced Engineering Materials 19(11) (2017).
- 1 2 X. Wang, X. Wei, C. Wen, F. Han, Fabrication and characterisation of microporous titanium, Powder metallurgy 54(1) (2011) 56-58.
- 1 2 3 4 5 6 7 N. Tuncer, G. Arslan, Designing compressive properties of titanium foams, Journal of materials science 44(6) (2009) 1477-1484.
- 1 2 3 4 N. Davis, J. Teisen, C. Schuh, D. Dunand, Solid-state foaming of titanium by superplastic expansion of argon-filled pores, Journal of Materials Research 16(5) (2001) 1508-1519.
- ↑ D. Elzey, H. Wadley, The limits of solid state foaming, Acta materialia 49(5) (2001) 849-859.
- ↑ L.P. Lefebvre, E. Baril, Effect of oxygen concentration and distribution on the compression properties on titanium foams, Advanced Engineering Materials 10(9) (2008) 868-876.
- 1 2 W.D. Callister, D.G. Rethwisch, Materials science and engineering: an introduction, Wiley New York2007.
- 1 2 3 4 C. Leyens, M. Peters, Titanium and titanium alloys, Wiley Online Library2003.
- 1 2 V. Srivastava, K. Sahoo, Processing, stabilization and applications of metallic foams. Art of science, Materials Science-Poland 25(3) (2007) 733-753.
- ↑ L. Zhang, Y. Zhao, Fabrication of high melting-point porous metals by lost carbonate sintering process via decomposition route, Proceedings of the Institution of Mechanical Engineers, Part B: Journal of Engineering Manufacture 222(2) (2008) 267-271.
- 1 2 A. Kennedy, S. Asavavisitchai, Effects of TiB 2 particle addition on the expansion, structure and mechanical properties of PM Al foams, Scripta Materialia 50(1) (2004) 115-119.
- 1 2 3 4 M. Sharma, G. Gupta, O. Modi, B. Prasad, PM processed titanium foam: influence of morphology and content of space holder on microstructure and mechanical properties, Powder Metallurgy 56(1) (2013) 55-60.
- ↑ N. Murray, D. Dunand, Microstructure evolution during solid-state foaming of titanium, Composites science and technology 63(16) (2003) 2311-2316.
- 1 2 E.D. Spoerke, N.G. Murray, H. Li, L.C. Brinson, D.C. Dunand, S.I. Stupp, A bioactive titanium foam scaffold for bone repair, Acta Biomaterialia 1(5) (2005) 523-533.
- 1 2 V. Amigó Borrás, L. Reig Cerdá, D.J. Busquets Mataix, J. Ortiz, Analysis of bending strength of porous titanium processed by space holder method, (2011).
- ↑ Y. An, N. Oh, Y. Chun, Y. Kim, D. Kim, J. Park, J. Kwon, K. Choi, T. Eom, T. Byun, Mechanical properties of environmental-electro-discharge-sintered porous Ti implants, Materials letters 59(17) (2005) 2178-2182.
- ↑ O. Andersen, U. Waag, L. Schneider, G. Stephani, B. Kieback, Novel metallic hollow sphere structures, Advanced Engineering Materials 2(4) (2000) 192-195.
- ↑ M. Bram, H. Schiefer, D. Bogdanski, M. Köller, H. Buchkremer, D. Stöver, Implant surgery: How bone bonds to PM titanium, Metal Powder Report 61(2) (2006) 26-31.
- ↑ M. Bram, C. Stiller, H.P. Buchkremer, D. Stöver, H. Baur, High‐Porosity Titanium, Stainless Steel, and Superalloy Parts, Advanced Engineering Materials 2(4) (2000) 196-199.
- ↑ E. Carreño-Morelli, M. Rodríguez-Arbaizar, A. Amherd, J.-E. Bidaux, Porous titanium processed by powder injection moulding of titanium hydride and space holders, Powder Metallurgy 57(2) (2014) 93-96.
- ↑ Y. Chino, D.C. Dunand, Creating Aligned, Elongated Pores in Titanium Foams by Swaging of Preforms with Ductile Space‐Holder, Advanced Engineering Materials 11(1‐2) (2009) 52-55.
- ↑ X. Fan, B. Feng, Y. Di, J. Wang, X. Lu, J. Weng, Graded porous titanium scaffolds fabricated using powder metallurgy technique, Powder Metallurgy and Metal Ceramics 51(5-6) (2012) 372-377.
- ↑ T. Hong, Z. Guo, R. Yang, Fabrication of porous titanium scaffold materials by a fugitive filler method, Journal of Materials Science: Materials in Medicine 19(12) (2008) 3489-3495.
- ↑ A. Ibrahim, F. Zhang, E. Otterstein, E. Burkel, Processing of porous Ti and Ti5Mn foams by spark plasma sintering, Materials & Design 32(1) (2011) 146-153.
- 1 2 T. Imwinkelried, Mechanical properties of open‐pore titanium foam, Journal of biomedical materials research Part A 81(4) (2007) 964-970.
- ↑ C. Jee, Z. Guo, J. Evans, N. Özgüven, Preparation of high porosity metal foams, Metallurgical and Materials Transactions B 31(6) (2000) 1345-1352.
- 1 2 S. Kashef, J. Lin, P.D. Hodgson, W. Yan, Mechanical properties of titanium foam for biomedical applications, International journal of modern physics B 22(31n32) (2008) 6155-6160.
- ↑ A. Kostornov, S. Agayan, Determining the quality of baking of porous titanium to porosity-free, Powder Metallurgy and Metal Ceramics 29(10) (1990) 804-807.
- ↑ A. Kostornov, L. Galstyan, S. Mnatsakanyan, S. Agayan, The physicomechanical properties of porous titanium base powder materials, Powder Metallurgy and Metal Ceramics 25(11) (1986) 909-911.
- ↑ P.J. Kwok, S.M. Oppenheimer, D.C. Dunand, Porous Titanium by Electro‐chemical Dissolution of Steel Space‐holders, Advanced Engineering Materials 10(9) (2008) 820-825.
- 1 2 A. Laptev, M. Bram, H. Buchkremer, D. Stöver, Study of production route for titanium parts combining very high porosity and complex shape, Powder metallurgy 47(1) (2004) 85-92.
- ↑ A. Laptev, O. Vyal, M. Bram, H. Buchkremer, D. Stöver, Green strength of powder compacts provided for production of highly porous titanium parts, Powder metallurgy 48(4) (2005) 358-364.
- ↑ B. Lee, T. Lee, Y. Lee, D.J. Lee, J. Jeong, J. Yuh, S.H. Oh, H.S. Kim, C.S. Lee, Space-holder effect on designing pore structure and determining mechanical properties in porous titanium, Materials & Design 57 (2014) 712-718.
- ↑ A. Manonukul, N. Muenya, F. Leaux, S. Amaranan, Effects of replacing metal powder with powder space holder on metal foam produced by metal injection moulding, Journal of Materials Processing Technology 210(3) (2010) 529-535.
- ↑ A. Manonukul, M. Tange, P. Srikudvien, N. Denmud, P. Wattanapornphan, Rheological properties of commercially pure titanium slurry for metallic foam production using replica impregnation method, Powder Technology 266 (2014) 129-134.
- 1 2 A. Mansourighasri, N. Muhamad, A. Sulong, Processing titanium foams using tapioca starch as a space holder, Journal of Materials Processing Technology 212(1) (2012) 83-89.
- 1 2 D. Mondal, J. Datta Majumder, N. Jha, A. Badkul, S. Das, A. Patel, G. Gupta, Titanium-cenosphere syntactic foam made through powder metallurgy route, Materials & Design 34 (2012) 82-89.
- ↑ D. Mondal, M. Patel, S. Das, A. Jha, H. Jain, G. Gupta, S. Arya, Titanium foam with coarser cell size and wide range of porosity using different types of evaporative space holders through powder metallurgy route, Materials & Design 63 (2014) 89-99.
- 1 2 3 W. Niu, C. Bai, G. Qiu, Q. Wang, Processing and properties of porous titanium using space holder technique, Materials Science and Engineering: A 506(1) (2009) 148-151.
- ↑ W. Niu, S. Gill, H. Dong, C. Bai, A two-scale model for predicting elastic properties of porous titanium formed with space-holders, Computational Materials Science 50(1) (2010) 172-178.
- 1 2 3 4 5 M. Sharma, G. Gupta, O. Modi, B. Prasad, A.K. Gupta, Titanium foam through powder metallurgy route using acicular urea particles as space holder, Materials Letters 65(21) (2011) 3199-3201.
- ↑ R. Singh, P. Lee, T. Lindley, C. Kohlhauser, C. Hellmich, M. Bram, T. Imwinkelried, R. Dashwood, Characterization of the deformation behavior of intermediate porosity interconnected Ti foams using micro-computed tomography and direct finite element modeling, Acta biomaterialia 6(6) (2010) 2342-2351.
- 1 2 R. Singh, P. Lee, T.C. Lindley, R. Dashwood, E. Ferrie, T. Imwinkelried, Characterization of the structure and permeability of titanium foams for spinal fusion devices, Acta Biomaterialia 5(1) (2009) 477-487.
- 1 2 3 O. Smorygo, A. Marukovich, V. Mikutski, A. Gokhale, G.J. Reddy, J.V. Kumar, High-porosity titanium foams by powder coated space holder compaction method, Materials Letters 83 (2012) 17-19.
- ↑ Y. Torres, S. Lascano, J. Bris, J. Pavón, J.A. Rodriguez, Development of porous titanium for biomedical applications: A comparison between loose sintering and space-holder techniques, Materials Science and Engineering: C 37 (2014) 148-155.
- ↑ Y. Torres, J. Rodríguez, S. Arias, M. Echeverry, S. Robledo, V. Amigo, J. Pavón, Processing, characterization and biological testing of porous titanium obtained by space-holder technique, Journal of Materials Science 47(18) (2012) 6565-6576.
- ↑ L. Tuchinskiy, R. Loutfy, Titanium foams for medical applications, Medical Device Materials: Proceedings from the Materials & Processes for Medical Devices Conference 2003, 8–10 September 2003, Anaheim, California, ASM International, 2004, p. 377.
- 1 2 3 N. Tuncer, G. Arslan, E. Maire, L. Salvo, Influence of cell aspect ratio on architecture and compressive strength of titanium foams, Materials Science and Engineering: A 528(24) (2011) 7368-7374.
- 1 2 3 4 5 N. Tuncer, G. Arslan, E. Maire, L. Salvo, Investigation of spacer size effect on architecture and mechanical properties of porous titanium, Materials Science and Engineering: A 530 (2011) 633-642.
- 1 2 3 4 5 6 C. Wen, M. Mabuchi, Y. Yamada, K. Shimojima, Y. Chino, T. Asahina, Processing of biocompatible porous Ti and Mg, Scripta Materialia 45(10) (2001) 1147-1153.
- 1 2 3 4 5 6 C. Wen, Y. Yamada, K. Shimojima, Y. Chino, H. Hosokawa, M. Mabuchi, Novel titanium foam for bone tissue engineering, Journal of materials research 17(10) (2002) 2633-2639.
- 1 2 3 4 5 B. Ye, D.C. Dunand, Titanium foams produced by solid-state replication of NaCl powders, Materials Science and Engineering: A 528(2) (2010) 691-697.
- 1 2 F. Zhang, E. Otterstein, E. Burkel, Spark plasma sintering, microstructures, and mechanical properties of macroporous titanium foams, Advanced Engineering Materials 12(9) (2010) 863-872.
- ↑ N. Murray, C. Schuh, D. Dunand, Solid-state foaming of titanium by hydrogen-induced internal-stress superplasticity, Scripta Materialia 49(9) (2003) 879-883.
- ↑ Y. Zhao, D. Sun, A novel sintering-dissolution process for manufacturing Al foams, Scripta materialia 44(1) (2001) 105-110.
- 1 2 N. Jha, D. Mondal, J.D. Majumdar, A. Badkul, A. Jha, A. Khare, Highly porous open cell Ti-foam using NaCl as temporary space holder through powder metallurgy route, Materials & Design 47 (2013) 810-819
- 1 2 3 4 Y. Zhao, Porous Metallic Materials Produced by P/M Methods, Journal of Powder Metallurgy & Mining (2013).
- 1 2 3 A. Mansourighasri, N. Muhamad, A.B. Sulong, Processing titanium foams using tapioca starch as a space holder, Journal of Materials Processing Technology 212(1) (2012) 83-89.
- 1 2 G. Ryan, A. Pandit, D.P. Apatsidis, Fabrication methods of porous metals for use in orthopaedic applications, Biomaterials 27(13) (2006) 2651-2670.
- 1 2 3 4 5 6 A. Bansiddhi, T. Sargeant, S. Stupp, D. Dunand, Porous NiTi for bone implants: a review, Acta biomaterialia 4(4) (2008) 773-782.
- ↑ J.C. Li, D.C. Dunand, Mechanical properties of directionally freeze-cast titanium foams, Acta Materialia 59(1) (2011) 146-158.
- ↑ A. Bansiddhi, D. Dunand, Shape-memory NiTi foams produced by replication of NaCl space-holders, Acta biomaterialia 4(6) (2008) 1996-2007.
- ↑ A. Bansiddhi, D.C. Dunand, Shape-memory NiTi–Nb foams, Journal of Materials Research 24(06) (2009) 2107-2117.
- ↑ L.-J. Chen, T. Li, Y.-M. Li, H. He, Y.-H. Hu, Porous titanium implants fabricated by metal injection molding, Transactions of Nonferrous Metals Society of China 19(5) (2009) 1174-1179.
- ↑ X. Zhao, H. Sun, L. Lan, J. Huang, H. Zhang, Y. Wang, Pore structures of high-porosity NiTi alloys made from elemental powders with NaCl temporary space-holders, Materials Letters 63(28) (2009) 2402-2404.
- ↑ Y. Torres, J. Pavón, J. Rodríguez, Processing and characterization of porous titanium for implants by using NaCl as space holder, Journal of Materials Processing Technology 212(5) (2012) 1061-1069.
- ↑ A. Bansiddhi, D.C. Dunand, Shape-memory NiTi foams produced by replication of NaCl space-holders, Acta Biomaterialia 4(6) (2008) 1996-2007.
- ↑ A. Bansiddhi, D.C. Dunand, Shape-memory NiTi foams produced by solid-state replication with NaF, Intermetallics 15(12) (2007) 1612-1622.
- ↑ T. Aydoğmuş, Ş. Bor, Processing of porous TiNi alloys using magnesium as space holder, Journal of alloys and compounds 478(1) (2009) 705-710.
- 1 2 S.W. Kim, H.-D. Jung, M.-H. Kang, H.-E. Kim, Y.-H. Koh, Y. Estrin, Fabrication of porous titanium scaffold with controlled porous structure and net-shape using magnesium as spacer, Materials Science and Engineering: C 33(5) (2013) 2808-2815.
- ↑ G. Kotan, A.Ş. Bor, Production and characterization of high porosity Ti-6Al-4V foam by space holder technique in powder metallurgy, Turkish Journal of Engineering and Environmental Sciences 31(3) (2007) 149-156.
- ↑ K. Scotti, FreezeCasting.net Open-Data Repository, 2017.
- 1 2 K. Scotti, D. Dunand, Freeze Casting: A Review of Processing, Microstructure and Properties via the Open Data Repository, FreezeCasting. net, arXiv preprint arXiv:1710.00037 (2017).
- ↑ P. Jenei, H. Choi, A. Tóth, H. Choe, J. Gubicza, Mechanical behavior and microstructure of compressed Ti foams synthesized via freeze casting, J. Mech. Behav. Biomed. Mater. (2016).
- ↑ H. Jung, S. Yook, T. Jang, Y. Li, H. Kim, Y. Koh, Dynamic freeze casting for the production of porous titanium (Ti) scaffolds, Mater. Sci. Eng. C 33(1) (2013) 59-63.
- ↑ H. Lee, T. Jang, J. Song, H. Kim, H. Jung, Multi-scale porous Ti6Al4V scaffolds with enhanced strength and biocompatibility formed via dynamic freeze-casting coupled with micro-arc oxidation, Mater. Lett. 185 (2016) 21-24.
- ↑ S. Yook, H. Jung, C. Park, K. Shin, Y. Koh, Y. Estrin, H. Kim, Reverse freeze casting: A new method for fabricating highly porous titanium scaffolds, with aligned large pores, Acta Biomater. 8(6) (2012) 2401-2410.
- ↑ S. Yook, H. Kim, Y. Koh, Fabrication of porous titanium scaffolds with high compressive strength using camphene-based freeze casting, Mater. Lett. 63(17) (2009) 1502-1504.
- ↑ S. Yook, B. Yoon, H. Kim, Y. Koh, Y. Kim, Porous titanium (Ti) scaffolds by freezing TiH2/camphene slurries, Mater. Lett. 62(30) (2008) 4506-4508.
- ↑ Y. Chino, D.C. Dunand, Directionally freeze-cast titanium foam with aligned, elongated pores, Acta Mater. 56(1) (2008) 105-113.
- ↑ Li, J. C., & Dunand, D. C. (2011). Mechanical properties of directionally freeze-cast titanium foams. Acta Materialia, 59(1), 146-158.
- ↑ Fife, J. L., Li, J. C., Dunand, D. C., & Voorhees, P. W. (2009). Morphological analysis of pores in directionally freeze-cast titanium foams. Journal of Materials Research, 24(1), 117-124.
- ↑ Weaver, J. S., Kalidindi, S. R., & Wegst, U. G. (2017). Structure-processing correlations and mechanical properties in freeze-cast Ti-6Al-4V with highly aligned porosity and a lightweight Ti-6Al-4V-PMMA composite with excellent energy absorption capability. Acta Materialia, 132, 182-192.
- ↑ Y. Zhao, T. Fung, L. Zhang, F. Zhang, Lost carbonate sintering process for manufacturing metal foams, Scripta Materialia 52(4) (2005) 295-298.
- 1 2 3 S. Yang, C. Qi, D. Wang, R. Gao, H. Hu, J. Shu, A Comparative Study of Ballistic Resistance of Sandwich Panels with Aluminum Foam and Auxetic Honeycomb Cores, Advances in Mechanical Engineering 2013 (2013).
- ↑ K. Finnegan, G. Kooistra, H.N. Wadley, V. Deshpande, The compressive response of carbon fiber composite pyramidal truss sandwich cores, International Journal of Materials Research 98(12) (2007) 1264-1272.
- ↑ S. Park, B. Russell, V. Deshpande, N. Fleck, Dynamic compressive response of composite square honeycombs, Composites Part A: Applied Science and Manufacturing 43(3) (2012) 527-536.
- ↑ F. Zok*, H. Rathbun, M. He, E. Ferri, C. Mercer, R. McMeeking, A. Evans, Structural performance of metallic sandwich panels with square honeycomb cores, Philosophical Magazine 85(26-27) (2005) 3207-3234.
- 1 2 H. Rathbun, D. Radford, Z. Xue, M. He, J. Yang, V. Deshpande, N. Fleck, J. Hutchinson, F. Zok, A. Evans, Performance of metallic honeycomb-core sandwich beams under shock loading, International journal of solids and structures 43(6) (2006) 1746-1763.
- ↑ J. Luyten, S. Mullens, I. Thijs, Designing with pores-synthesis and applications, KONA Powder and Particle Journal 28 (2010) 131-142.
- ↑ P. Moongkhamklang, D.M. Elzey, H.N. Wadley, Titanium matrix composite lattice structures, Composites Part A: applied science and manufacturing 39(2) (2008) 176-187.
- ↑ P. Moongkhamklang, V. Deshpande, H. Wadley, The compressive and shear response of titanium matrix composite lattice structures, Acta Materialia 58(8) (2010) 2822-2835.
- ↑ A. Alderson, K. Alderson, Auxetic materials, Proceedings of the Institution of Mechanical Engineers, Part G: Journal of Aerospace Engineering 221(4) (2007) 565-575.
- ↑ A. Alderson, J. Rasburn, S. Ameer-Beg, P.G. Mullarkey, W. Perrie, K.E. Evans, An auxetic filter: a tuneable filter displaying enhanced size selectivity or defouling properties, Industrial & engineering chemistry research 39(3) (2000) 654-665.
- ↑ F. Scarpa, L. Ciffo, J. Yates, Dynamic properties of high structural integrity auxetic open cell foam, Smart materials and structures 13(1) (2004) 49.
- 1 2 A. Alderson, A triumph of lateral thought, Chem. Ind 10 (1999) 384.
- 1 2 C. Elias, J. Lima, R. Valiev, M. Meyers, Biomedical applications of titanium and its alloys, Jom 60(3) (2008) 46-49.
- ↑ H. Agins, N. Alcock, M. Bansal, E. Salvati, P. Wilson, P. Pellicci, P. Bullough, Metallic wear in failed titanium-alloy total hip replacements, J Bone Joint Surg 70(3) (1988) 347-356.
- ↑ D.N. Caborn, W.P. Urban, D.L. Johnson, J. Nyland, D. Pienkowski, Biomechanical comparison between BioScrew and titanium alloy interference screws for bone—patellar tendon—bone graft fixation in anterior cruciate ligament reconstruction, Arthroscopy: The Journal of Arthroscopic & Related Surgery 13(2) (1997) 229-232.
- ↑ M. Culjat, R. Singh, H. Lee, Medical devices: surgical and image-guided technologies, John Wiley & Sons2012.
- ↑ M. Long, H. Rack, Titanium alloys in total joint replacement—a materials science perspective, Biomaterials 19(18) (1998) 1621-1639.
- ↑ D.D. Deligianni, N. Katsala, S. Ladas, D. Sotiropoulou, J. Amedee, Y. Missirlis, Effect of surface roughness of the titanium alloy Ti–6Al–4V on human bone marrow cell response and on protein adsorption, Biomaterials 22(11) (2001) 1241-1251.
- ↑ M. Donachie, Titanium: a technical guide, ASM international2000.
- ↑ H.L. Freese, M.G. Volas, J.R. Wood, Metallurgy and technological properties of titanium and titanium alloys, Titanium in Medicine, Springer2001, pp. 25-51.
- ↑ A. Holton, E. Walsh, A. Anayiotos, G. Pohost, R. Venugopalan, Comparative MRI Compatibility of 316L Stainless Steel Alloy and Nickel–Titanium Alloy Stents: Original article Technical, Journal of Cardiovascular Magnetic Resonance 4(4) (2002) 423-430.
- ↑ A. Malik, O. Boyko, N. Aktar, W. Young, A comparative study of MR imaging profile of titanium pedicle screws, Acta Radiologica 42(3) (2001) 291-293.
- ↑ O. Ortiz, T.G. Pait, P. McAllister, K. Sauter, Postoperative magnetic resonance imaging with titanium implants of the thoracic and lumbar spine, Neurosurgery 38(4) (1996) 741-745.
- ↑ J. Jakubowicz, G. Adamek, M. Dewidar, Titanium foam made with saccharose as a space holder, Journal of Porous Materials 20(5) (2013) 1137-1141.
- 1 2 K. Abshagen, I. Schrodi, T. Gerber, B. Vollmar, In vivo analysis of biocompatibility and vascularization of the synthetic bone grafting substitute NanoBone®, Journal of Biomedical Materials Research Part A 91(2) (2009) 557-566.
- ↑ I.-H. Oh, N. Nomura, N. Masahashi, S. Hanada, Mechanical properties of porous titanium compacts prepared by powder sintering, Scripta Materialia 49(12) (2003) 1197-1202.
- ↑ C. Greiner, S.M. Oppenheimer, D.C. Dunand, High strength, low stiffness, porous NiTi with superelastic properties, Acta Biomaterialia 1(6) (2005) 705-716.
- ↑ M.S. Aly, Effect of pore size on the tensile behavior of open-cell Ti foams: Experimental results, Materials letters 64(8) (2010) 935-937.
- 1 2 L.E. Murr, S.M. Gaytan, E. Martinez, F. Medina, R.B. Wicker, Next generation orthopaedic implants by additive manufacturing using electron beam melting, International journal of biomaterials 2012 (2012).
- 1 2 3 H. Li, S.M. Oppenheimer, S.I. Stupp, D.C. Dunand, L.C. Brinson, Effects of pore morphology and bone ingrowth on mechanical properties of microporous titanium as an orthopaedic implant material, Materials Transactions 45(4) (2004) 1124-1131.
- ↑ Y. Conde, A. Pollien, A. Mortensen, Functional grading of metal foam cores for yield-limited lightweight sandwich beams, Scripta Materialia 54(4) (2006) 539-543.
- ↑ H.-W. Kim, Y.-H. Koh, L.-H. Li, S. Lee, H.-E. Kim, Hydroxyapatite coating on titanium substrate with titania buffer layer processed by sol–gel method, Biomaterials 25(13) (2004) 2533-2538.
- ↑ R.M. Wazen, L.P. Lefebvre, E. Baril, A. Nanci, Initial evaluation of bone ingrowth into a novel porous titanium coating, Journal of Biomedical Materials Research Part B: Applied Biomaterials 94(1) (2010) 64-71.
- ↑ V.D. Rani, K. Manzoor, D. Menon, N. Selvamurugan, S.V. Nair, The design of novel nanostructures on titanium by solution chemistry for an improved osteoblast response, Nanotechnology 20(19) (2009) 195101.
- ↑ B.K. Nayak, M.C. Gupta, Self-organized micro/nano structures in metal surfaces by ultrafast laser irradiation, Optics and Lasers in Engineering 48(10) (2010) 940-949.
- ↑ R. Karpagavalli, A. Zhou, P. Chellamuthu, K. Nguyen, Corrosion behavior and biocompatibility of nanostructured TiO2 film on Ti6Al4V, Journal of Biomedical Materials Research Part A 83(4) (2007) 1087-1095
- ↑ D.W. Hutmacher, Scaffolds in tissue engineering bone and cartilage, Biomaterials 21(24) (2000) 2529-2543
- 1 2 S.N. Khan, M. Ramachandran, S.S. Kumar, V. Krishnan, R. Sundaram, Osseointegration and more–A review of literature, Indian Journal of Dentistry 3(2) (2012) 72-76.
- ↑ C. Leyens, M. Peters, Titanium and titanium alloys: fundamentals and applications, John Wiley & Sons2003.
- ↑ M. Lutolf, J. Hubbell, Synthetic biomaterials as instructive extracellular microenvironments for morphogenesis in tissue engineering, Nature biotechnology 23(1) (2005) 47.
- ↑ Z. Tang, N.A. Kotov, S. Magonov, B. Ozturk, Nanostructured artificial nacre, Nature materials 2(6) (2003) 413
- ↑ F. Heinemann, T. Mundt, R. Biffar, T. Gedrange, W. Goetz, A 3-year clinical and radiographic study of implants placed simultaneously with maxillary sinus floor augmentations using a new nanocrystalline hydroxyapatite, Journal of Physiology and Pharmacology 60(supplement 8) (2009) 91-97.
- ↑ B.M. Willie, X. Yang, N.H. Kelly, J. Merkow, S. Gagne, R. Ware, T.M. Wright, M.P. Bostrom, Osseointegration into a novel titanium foam implant in the distal femur of a rabbit, Journal of Biomedical Materials Research Part B: Applied Biomaterials 92(2) (2010) 479-488